Program of research
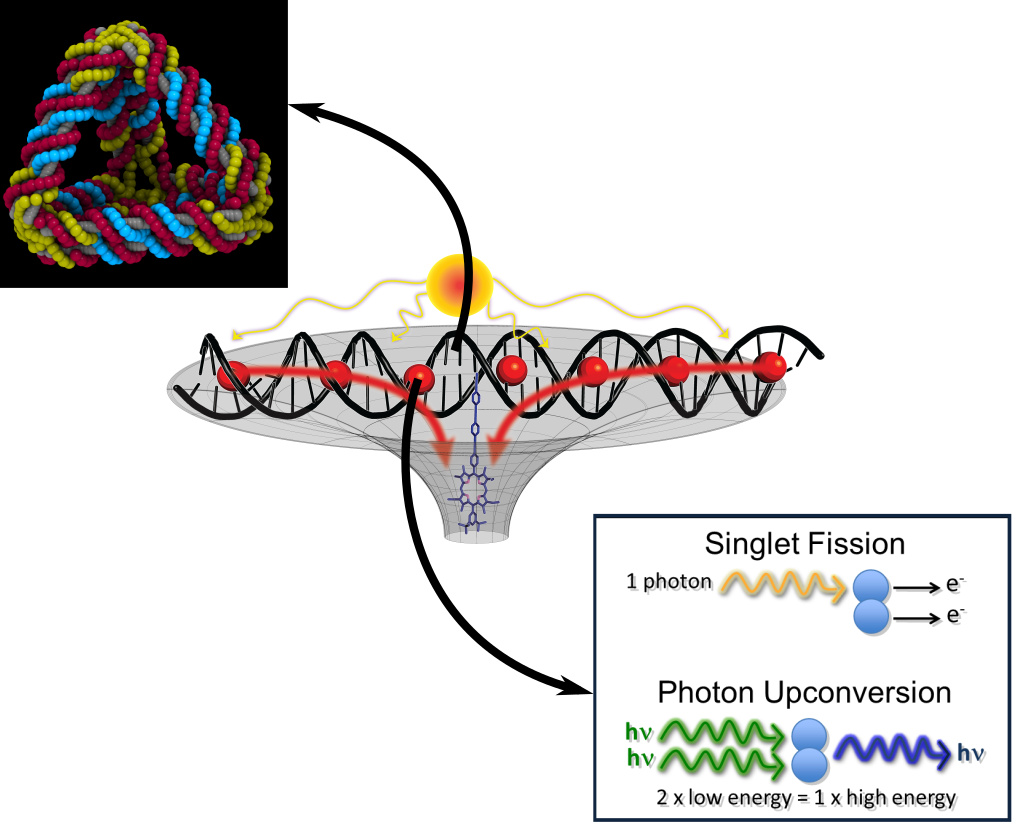
My multidisciplinary research program is at the intersection of physical chemistry, photochemistry, and biophysics. I'm primarily interested in understanding, characterising, and optimising the energy transfer through molecules and supramolecular complexes. These molecular systems will be applied to light-harvesting devices to enhance their solar-collection efficiency. Solar cells and other photonic applications are prime targets for this research. A representative 'synthetic leaf' design is shown in Figure 1.
Understanding and controlling the fundamentals of molecular coupling are essential in selecting the mode and efficiency of energy transfer among a molecular network. My group will exploit non-linear energy transfer processes, such as upconversion and singlet-splitting, which combine or generate multiple photons on ultrafast timescales. These non-linear features enable the molecular systems to boost low-energy light to higher energies and overcome fundamental limitations in light-harvesting technologies.
For these modes of energy transfer to occur, it is crucial that the molecular coupling is tuned by the appropriate intermolecular orientations and distances. To fix these parameters, we will utilise DNA origami techniques to prepare tailor-made scaffolds to which the molecules will be bound.
To test the efficiency of energy transfer amongst the molecules in these biomolecular complexes, we will harness our ultrafast laser-based spectroscopy expertise.
In addition, we are privileged to work with collaborators across Canada, and beyond, to characterise the photophysics and chemistry of their innovative molecular samples. The results of our experiments can help our colleagues understand light-triggered chemical processes and suggest sample improvements.
Continue reading for more details.
Non-linear energy transfer modes
One aspect of my group's research is the investigation of molecules capable of extremely efficient energy transfer. Ideally the molecules should have properties that can be altered easily by the addition of side chains or the replacement of functional groups. Hence, I focus on organic molecules, especially conjugated aromatic structures.
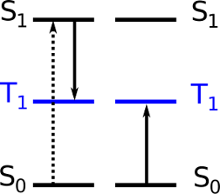
Ideal chromophores are those that absorb and emit light in the visible spectrum and undergo non-linear energy transfer processes. One such process is singlet fission, in which a high-energy UV photon is absorbed by a molecule's first singlet excited state and converted into two lower-energy triplet photons, shown in Figure 2. (A molecule's singlet and triplet states refer to the different ways in which electrons can pair in these states, i.e. their multiplicity.) This photon multiplication process combats the maximum theoretical efficiency of a solar cell with a single junction (the Shockley-Queisser limit), and is potentially very fast and efficient as the transition is 'allowed'. These features make it an attractive process for technological applications. However, singlet fission is still not a well-understood process, due to the lack of molecules that undergo this process because of stringent energy requirements of the participating energy states. We propose that by considering molecules that absorb energy in upper singlet states, a huge breakthrough in the availability of singlet-fission molecules and in understanding the singlet fission process would ensue.
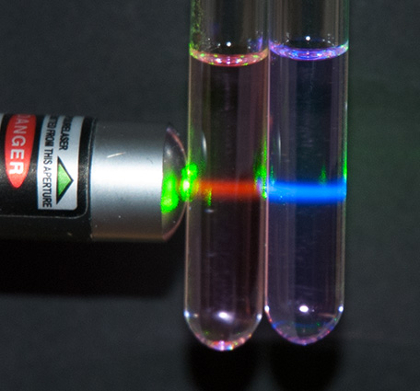
Several considerations must be addressed to ensure singlet fission is the predominant energy transfer pathway. The molecules must have at least two excitation sites next to each other to accommodate two triplet photons. Therefore, they must aggregate or couple with an appropriate strength so that they interact effectively, but without substantially altering their singlet or triplet state energies. The coupling can be controlled by altering the distance and orientation of the interacting molecules.
We have already explored these effects in the singlet fission reverse process. This process is known as upconversion or, more properly, triplet-triplet annihilation noncoherent photon upconversion and is illustrated in Figure 3. Here, a green laser pointer is exciting two molecular solutions in test tubes. In the left test tube, the molecules absorb the green light and emit red fluorescence. The red fluorescence is lower energy than the absorbing light as the molecules dissipate some of their vibrational energy to their solvent. In contrast, the molecules in the right test tube absorb the green light and upconvert it into higher-energy blue fluorescence. While we seem to be getting higher energy light for 'free', this is not actually the case as upconversion has at most an efficiency of 50%.
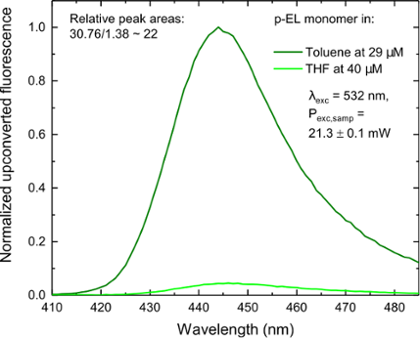
We have studied upconversion in porphyrins, most commonly zinc tetraphenyl porphyrins (ZnTPP), as they resemble chlorophylls, which are the light-harvesting pigments in plants. ZnTPP also readily upconverts absorbed light without the need for another participating molecule. This keeps our results as easy to interpret as possible.
In Figure 4 we see the upconverted signals from slightly modified ZnTPP molecules in two different solvents. Here, two of the molecule are excited into their singlet (S1) states with green light, with a wavelength of 532 nm (λexc). These states convert into triplet states via intersystem crossing. When two of these molecules approach one another closely enough the triplets are annihilated, with one molecule going back into its ground state and the other being excited into its upper singlet state (S2, for zinc porphyrins). Thus, the resulting emission signal shown in the graph is fluorescence from the S2 state, which appears as a broad emission centred around 442 nm.
For upconversion to compete with other de-excitation processes, the rate constant for the overall process must be large. This depends on multiple factors, which as for singlet fission, includes the distance and orientation of the excited species. In Figure 4, the solvent tetrahydrofuran (THF) coordinates to the zinc metal centres in the porphyrins and prevents the porphyrins approaching closely. As a result of the larger distance between the molecules in THF, as opposed to toluene, the efficiency of the porphyrin's upconversion process is decreased by a factor of 22.
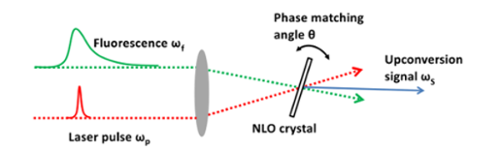
We use steady-state and ultrafast optical spectroscopy methods, such as upconversion spectroscopy and time-correlated single photon counting (TCSPC), to measure the fundamental properties and dynamics of molecules. Figure 5 shows an upconversion spectroscopy schematic. This technique employs non-linear optics (NLO) to measure signals on the femtosecond (10-15 seconds) or picosecond timescale (10-12 seconds). It is an ideal method to investigate the lifetimes of short-lived upper excited states (S2, S3) of porphyrins and azulenes.
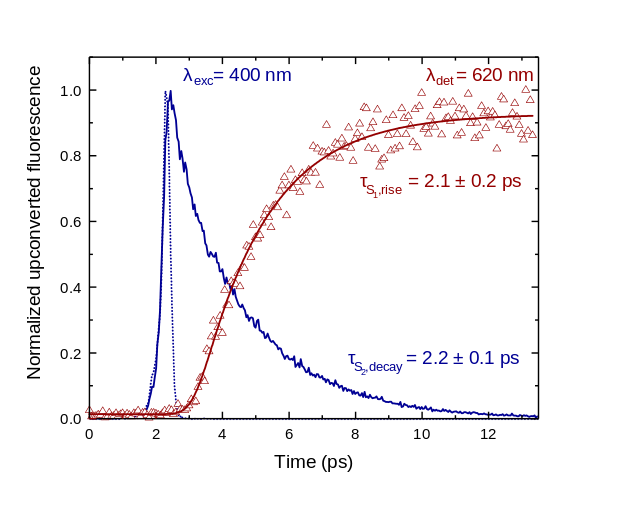
TCSPC has a nanosecond (10-9 seconds) timescale and utilises electronic methods, rather than non-linear optics, to measure fluorescence signals. With this, we can determine the lifetimes of longer-lived lower energy states (S1, etc).
Figure 6 shows the result of an upconversion spectroscopy experiment. The energy from the upper-excited states (S2) of the zinc porphyrins transfers to their lower energy (S1) states in 2 picoseconds. If the decay time becomes shorter, for example, we know that there are extra de-excitation processes occurring and we can start to investigate what these processes might be.
With our suite of tools and instruments available, listed in a further section, we will be able to assess the viability of azulenes and azulene-derivatives as a new class of singlet-fission materials and suggest chemical alterations to enhance their energy-transfer efficiency.
Multichromophoric arrays
The second and complimentary aspect of my group's research is to direct the arrangement of chromophores into a supramolecular array. This is crucial to realize their full energy-transfer potential and form a viable biomaterial capable of being incorporated into real-world light-harvesting applications.
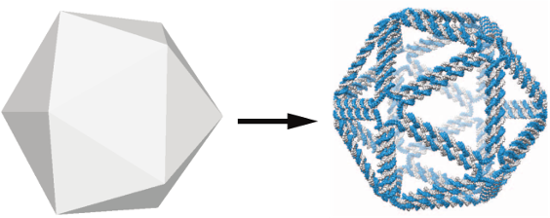
For optimum light-absorption efficiency, we must incorporate a large number of molecular chromophores in a small volume. We also need to avoid concentration-quenching effects that occur as the distance between the chromophores decreases and they interact more strongly. Structures are needed to tightly control the position of the chromophores to negate detrimental interactions and promote the desired energy relaxation pathway, which in our case is energy transfer and singlet fission.
We will attempt to use DNA as the structural element of these supramolecular complexes. With the advent of DNA nanotechnology techniques, also known as DNA origami, DNA can self-assemble into a multitude of three-dimensional shapes, through its specific Watson-Crick interactions. Figure 7 shows a modelled 3-dimensional shape that is realised by a wireframe DNA structure.
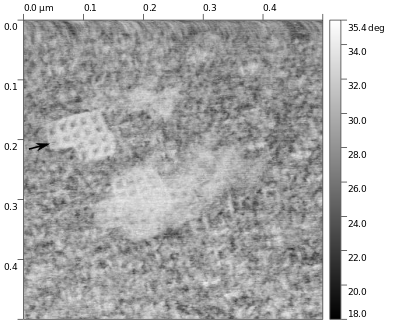
DNA can combine with chromophores in various ways, such as by intercalation between the base pairs of DNA, or binding to the major or minor grooves. Hence, the chromophores can be arranged in defined positions along the DNA strand, which sets their distance and orientation parameters.
To investigate the formation of these DNA nanostructures, we employ a variety of bioanalytical techniques, such as gel electrophoresis, atomic force microscopy (AFM), dynamic light scattering, and cryo-transmission electron microscopy. An AFM image of 'L-shaped' DNA that my group folded; based on the work of H. Jun et al, Sci Adv (2019) 5, 10398, eaav0655; is shown in Figure 8. Comparing the absorption, circular and linear dichroism, and decay kinetics results of the bare DNA structures to the chromophore-decorated DNA structures will test for effective chromophore incorporation. Our ultrafast spectroscopy methods will be used to investigate for the influence of the DNA environment on, the energy transfer among, and the energy delocalization across the coordinated chromophores.
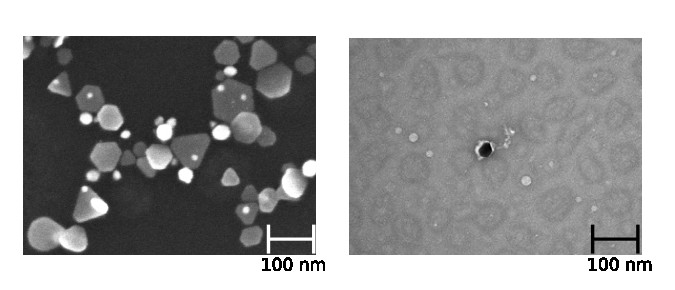
Recently, we have started preparing metal nanoparticles and combining them with our DNA origami structures. Figure 9 shows two transmission electron microscopy (TEM) images of these samples. On the left, we can see an image of our mostly triangular metal nanoparticles while, on the right, one of these nanoparticles is attached to a single strand of DNA. The DNA appears as a white squiggle structure to the right of the nanoparticle.
A full list of available steady-state and time-resolved spectroscopy equipment is listed in the next section.
Available equipment
Steady-state equipment: |
|
Absorption spectrophotometer (Cary 6000i) |
Fluorometer (Edinburgh Instruments) |
SPEX/homebuilt fluorometer with laser excitation |
Flash photolysis |
Fluorometer (PTI) |
Raman microscope - SSSC equipment |
Time-resolved equipment: |
|
Time-correlated single photon counting (TCSPC) lasers - SSSC equipment |
TCSPC sample compartment - SSSC equipment |
Upconversion lasers - SSSC equipment |
Upconversion laser path and detection optics - SSSC equipment |
Other: |
|
Vacuum line and vacuum sample holder for thin films |
TEM at vet med. |